ASTR 511 (O'Connell) Lecture Notes
CCD'S IN ASTRONOMY
Subaru CCD Mosaic 8 x (2k x 4k)
Charged coupled devices (CCD's) have been used in astronomy since the
late 1970's. They represent the third of the three most important
technical innovations for observational UVOIR astronomy in the second
half of the 20th century (the others being space telescopes
and interactive computing). They are now nearly ubiquitous in
observations made at wavelengths between the near-IR (~1 µ) and
the X-ray.
Sensitive 2-D area detectors like CCD's are critical not just for
astronomical imaging and photometry but for many other
state-of-the-art applications, including high precision astrometry
with Gaia
and large-scale, multi-object spectroscopic surveys (such as
the
Sloan Digital Sky Survey).
They have transformed the way astronomy is done.
I. REFERENCES FOR CCD'S
General
- Buil, CCD Astronomy
- Howell, Handbook of CCD Astronomy
- Kitchin, Astrophysical Techniques
- Rieke, Detection of Light : general introduction to detector physics
- LLM Sec. 7.3.3.
-
MacKay, Ann Rev Astr Ap, 24, 255, 1986 "CCDs in Astronomy" (review)
-
Timothy, PASP, 95, 810, 1983: discussion of many detector types
CCD Data Reduction and Applications
-
Abbott, "In
Situ CCD Testing"
- Massey, A User's Guide to CCD
Reductions with IRAF (1997)
- Craig & Chambers
(Astropy), CCD
Data Reduction Guide (Python)
- Tyson,
JOSAA, 3, 2131, 1986: high precision technique
-
Hook & Fruchter, "Dithering, Sampling and Image Reconstruction";
also see
the HST
DrizzlePak Handbook.
- Stetson,
PASP, 106, 250, 1994 and references therein. Widely-used ALLFRAME
and DAOPHOT point-source CCD photometry programs.
-
Franx et al. AJ, 98, 538, 1989: sample application to
surface photometry
-
Bertin & Arnouts, A&AS, 317, 393, 1996 SExtractor---source
ID and extraction software for digital images. Obtain software
here.
II. GENERAL DETECTOR CHARACTERIZATION
QUANTUM EFFICIENCY
- QE = percentage of photons incident on detector that produce
measurable signals
- Strong wavelength dependence (e.g. threshold cutoffs set
by work function/band gap)
- Typical peak values:
- Eye: 1-2%
- Photographic plate (Pg): 1-2%
- Photomultiplier tube (PMT): 20-30%
- CCD: 70-90%
- IR array [HgCdTe]: 30-50%
|
Schematic QE curves for various classes of
detector
|
- "Detective quantum efficiency" is defined to be
[(SNRout)/(SNRin)]2, where SNR
is the signal-to-noise ratio and "in" and "out" refer to the
input and output signals to/from the detector, respectively.
DQE combines basic QE with the noise introduced by the detector.
This quantity is really what matters in comparing detectors, but
it is so dependent on specific details of
operations/applications that it is rarely used.
SPECTRAL RANGE
- Wavelength region over which QE is
sufficient for operation
DYNAMIC RANGE
- Definition: ratio of maximum to minimum measurable signal
- E.g. maximum number of events in a CCD pixel is determined by
photoelectron "full well" capacity or digitization maximum (typically 2 bytes);
minimum is determined by dark current/readout noise
- Applies to a single exposure; effective dynamic range
can be increased with multiple exposures
- Typical values: 100 (Pg); 104 (CCD); 105 (PMT)
- Related concepts:
- Linear Range: range of signals for which [Output] = k x [Input],
where k is a constant. Generally smaller than the calibrateable range
- Threshold: minimum measurable signal. Influenced by detector
noise or other intrinsic characteristics (e.g. fog on Pg plates)
- Saturation point: level where detector response ceases to
increase with the signal
NOISE CHARACTERISTICS
RESOLUTION
- Temporal
- PMT: ~0.0001 ms
- CCD: ~10 ms per pixel
- Spatial (array detectors)
- Pixel = minimum measureable area of detector surface. Typically
10-50 µ on Pg or semiconductor types. Pixels are not
necessarily independent of one another.
- Resolution cell: according to the Nyquist criterion, the
resolution cell is 2x the size of the minimum
independent measurable area. For proper sampling of an image,
we need at least 2 pixels per resolution cell, but in practice there
may be many more. A lower pixel density implies "undersampled"
imaging. A significantly higher pixel density does not provide more
information and is a waste of pixels.
For best pixel matching in an imaging application, the Nyquist
criterion implies that 1 pixel should span ~ (FWHM)/2, where FWHM is
the full width half maximum of the point spread function.
- Spectral
- Most UVOIR detectors are broad-band and have inherently
poor spectral resolution. One must use external elements (filters,
gratings, photonics) to provide spectral resolution
- Exception: superconducting "3D" detectors, which measure photon energy
as well as position; current spectral resolution is
R ~ 100
III. BRIEF HISTORY OF ASTRONOMICAL DETECTORS
PHOTOGRAPHIC ASTRONOMY:
Photographic emulsions work by storing in AgBr crystals the
photoelectrons ejected following absorption of photons during exposure
to light. Chemical reactions during development cause the crystals to
precipitate grains of silver, which form a permanent image.
Film was the detector of choice for most applications in astronomy
from around 1900 to 1950 and for imaging until about 1980. It is
impossible to exaggerate its importance to the development of modern
astronomy. Even with relatively low QE, photographic plates
offered decisive advantages over visual observations:
- Very long exposure times (up to a week in early applications),
meaning limiting fluxes thousands of times fainter
- A permanent,
objective record of astronomical images and spectra
- Geometric stability -- important for astrometry and morphology
- Large formats for wide field surveys
- Extension of observations to EM bands beyond the
"visual" band. The classic "photographic" band is centered around 4500
Å. Astronomical photography ultimately covered the range
3200-10000 Å.
However, the photographic emulsion had serious limitations with which
astronomers had long struggled:
- It is relatively insensitive,
with QE ~ 1%.
- Pg materials have non-negligible thresholds (a minimum of ~4
photoelectrons per grain). "Reciprocity failure" refers to the fact
that the threshold exposure must be delivered within a certain
critical time period or the photoelectrons on a grain will be
lost.
- Pg materials have a strongly non-linear response function,
and limited dynamic range.
- These properties plus the use of
hard-to-control chemical processes for emulsion deposition &
development makes them very difficult to calibrate
quantitatively.
- Each individual plate (or at the best, each co-processed "batch" of
plates) has different response characteristics.
-
Conversion of data to quantitative form (e.g. with microdensitometers) is
slow and cumbersome.
For details on astronomical photography at low light levels, see
Smith & Hoag
1979, ARAA, 17, 43.
PHOTOELECTRIC ASTRONOMY TO 1980:
In 1907, Joel
Stebbins (UIll, UWisc) began testing various types
of photoelectronic detectors. These, such as selenium
phototubes, were largely experimental and temperamental, but use of PE
devices spread after the 1920's. World War II greatly accelerated
these technologies, with mass production of high quality vacuum tubes
and sensitive electronics for detection of faint signals.
The key design for astronomy was the
photomultiplier
tube (PMT), the first widely used example of which was the RCA
1P21. The initial detector in a PMT is a photo-emissive
cathode surface, made from alkali metal compounds, which ejects a
single electron in response to a photon absorption. A series of other
"secondary electron emissive" surfaces (the "dynode chain") amplifies
this into a burst of ~106-7 electrons and deposits this on
an anode. (See illustration below.)
PMT's require cooling to suppress dark current and were typically
operated at dry-ice temperatures (-78C). The photocathode QE's of
PMT's in the optical region reached 20-40% by the 1960's, with very
wide acceptance bands.
Although PMT's were initially operated in a "direct current" mode,
where the average induced current was measured, the pulse-like
character of PMT output led to the adoption in astronomy of the same
kinds of high speed digital electronics that had been developed
earlier for accelerator and nuclear physics. This kind of
"pulse-counting" operation offers excellent noise rejection and
permits the detection of individual photons from cosmic
sources.
For more details on PMT's and PMT-based photometers, see the articles
by Lallemand and Johnson in Astronomical Techniques, ed. W. A. Hiltner
(Stars & Stellar Systems Vol II).
PMT's became the workhorses of multicolor photometry and
spectrophotometry after 1950, e.g. the Johnson & Morgan broad-band UBV
system (see Lecture 14 for details). They
featured excellent linearity and stability, which implied an
unprecedented capacity for accurate calibration of photometric
measurements. PMT filter photometry routinely reached the 1% level
for flux calibration. In turn, this was responsible for an explosion
in quantitative astrophysics.
Despite their excellent performance and their broad wavelength
response, PMT's were single element devices. They were not
easily adaptable to 1-D, let alone 2-D, applications (such as
imaging), despite heroic efforts such as the
33-channel Palomar
Multichannel Photoelectric Spectrometer (Oke, 1969).
Around 1960, a plethora of efforts began to develop robust
1-D and 2-D electronic devices suitable for astronomy. A dozen
or so of these produced practical systems (e.g. the
electronographic film camera, the Secondary Electron Conduction
Vidicon, the Intensified Dissector Scanner, the Intensified Reticon
Scanner, the Image Photon Counting System, and the Multi-Anode
Microchannel Array detector).
Most of these employ some type
of image
intensifier vacuum tube as a key element. This technology was
developed for military night vision systems. Intensifiers produce
easily detectable light pulses in a 2-D image field from individual
incident photons by accelerating the photo-electrons to high energies
and/or producing a cascade of electrons, then focussing these on a
luminescent output screen. Disadvantages include the frequent use of
high voltages (e.g. 25 kV) and serious image blur which, however, can
be reduced by the use of pulse centroiding electronics. Fiber
optic input/output plates were commonly used with intensifiers after
1970.
Microchannel
plate (MCP) intensifiers have often been used in space astronomy
missions (including HST, GALEX, and FUSE).
SOLID STATE ARRAY DETECTORS
But by far the most successful 2-D devices for astronomy emerging in
the last 50 years have been solid
state, semiconductor arrays. These are based on
photo-conductive materials fabricated with embedded
microelectronic integrated circuits on thin wafers
by photolithography.
Although lower quality devices can be mass-produced by microchip
"foundries," professional grade detectors still need to be
custom-processed.
The image at the right shows a wafer containing a number
of co-fabricated CCDs and other devices.
Solid state arrays are now employed as astronomical detectors from the
X-ray to the far-infrared. The most widely used is
the Charge-Coupled
Device (CCD), which operates at wavelengths from the X-ray to
~1 µ. CCD's were invented at Bell Labs in 1969 by G. Smith and
W. Boyle (Nobel Prize, 2009). By the mid-70's, CCDs were being tested
as astronomical detectors. They did not come into widespread use
until ca. 1980, following extensive development efforts associated
with
the
Wide Field & Planetary Camera on the Hubble Space Telescope, led
by J. Westphal, J. Gunn, and C. R. Lynds.
IV. SEMICONDUCTORS
Semiconductors are crystalline materials which are not
normally good conductors of electricity but which can be made to
conduct under certain circumstances. The central useful property of
semiconductors employed in astronomy is that their
electrical
properties change significantly after absorption of photons.
BAND GAPS: The properties of semiconductors are manipulated by
changing the structure of their internal energy levels, which are
spread out into "bands" by the proximity of the component atoms of the
solid. The "valence" band, corresponding to the outermost electrons in
a normal, unexcited atom, is the lowest energy band where electrons
are able to move between ions. However, no net conduction occurs as
long as the band is full. Above this lie the "conduction" bands,
which are not filled, and where electrons will move freely in response
to external EM fields. The separation between the valence and first conduction band
is called the "band gap energy", E
g.
Different materials have a wide range of band gaps. "Conductors" have
a zero gap, meaning that electrons are always available to transfer
charge. "Insulators" have very large gaps, implying zero conduction
except under extreme circumstances. "Semiconductors" have
intermediate gaps.
Absorption of a photon can push a valence electron into the conduction
band and produce a potential electrical signal. The photon energy
must exceed E
g, which implies that there is a
maximum
wavelength for excitation given by:
Lammax = 12,400 Å/Eg(eV)
Obviously, materials with band gaps in the few eV range are of interest
as potential UVOIR detectors. Band gaps and max wavelengths for some
important materials are given in the following table:
Material |
Eg(eV) |
Lammax (Å) |
Pure Si |
1.1 |
11,300 |
GaAs |
1.43 |
8,670 |
InSb |
0.36 |
34,400 |
Hg1-xCdxTe |
1.55x |
8,000/x |
DOPING: The "elemental"
semiconductors are those elements in group IVa of
the Periodic Table
(including Si and Ge). These have four electrons in their valence
shells, half the maximum allowed. These are shared among the ions in
"co-valent bonds." There are many other types of "compound"
semiconductors, however, composed for instance of atoms from group
IIIa and Va of the Table; two of these are listed in the table
above.
The electrical properties of pure semiconductors can be dramatically
altered by adding ("doping with") small amounts (~1 part in
106) of an impurity. The result is called an "extrinsic"
semiconductor.
- n-type: a material with more than 4 valence electrons is added
(As, from group Va, in the illustration). The extra electrons
cannot be accommodated in the valence band and so occupy the conduction
band. They represent a persistent set of negative carriers
- p-type: a material with fewer than 4 valence electrons is
added (e.g. B, from group IIIa). This has one fewer electron than normal
and creates a small "vacuum" in the electron sea of the valence band.
This is called a "hole." As valence electrons shift to fill it, the
hole propagates like a positive charge in the opposite direction.
The holes represent a persistent set of positive carriers.
- In pure semiconductors, conduction electrons and holes can also
exist, if electrons are excited by thermal effects, for instance. But
they always occur in pairs. Electrons and holes in n- and p-type
materials, respectively, have no counterparts. Extrinsic material is
electrically neutral but is more responsive than pure materials to a
difference in electrical potential.
By adjusting the amount of doping, the band gap of the semiconductor
(donor/acceptor levels in diagram at right) can be customized. By
layering n/p regions, the response to applied potentials can be adjusted
to create a large variety of electronic devices.
|
n-type doping
p-type doping
Doping affects energy levels
|
Photons are primarily absorbed by electrons in the valence band.
For photon energies above E
g, the electron is boosted
to the conduction band, leaving a hole behind. If a positive
voltage is applied at one side of the semiconductor, the electron
will be attracted toward it while the hole will be repelled.
V. BASIC CCD DESIGN
Apart from
sensitivity, the key design issues for solid state arrays
are to
localize photon-produced charges on their surfaces and then
arrange to
amplify and read them out without distorting the image or
introducing unacceptable amounts of noise.
A CCD is a
charge-transfer device. Its storage, transfer, and
amplification electronics are all fabricated on a single piece of
silicon. During an exposure, it traps released photoelectrons in
small voltage wells. After the exposure, the electrons are shifted in
a series of "charge-coupled" steps across the CCD surface, amplified,
read out of the CCD, and stored in a computer memory. This
is
"destructive readout" --i.e. one cannot read the same
signal again (unlike other array architectures, where each pixel is
coupled to a separate amplifier).
BASIC STRUCTURAL ELEMENT: The basic
element in a CCD design is a
"Metal-Oxide-Semiconductor" capacitor. See the illustration
above. This serves both to store photoelectrons and to shift them
wholesale. The bulk material is p-silicon on which an insulating
layer of silicon-oxide has been grown. P-silicon can be made to have
very few free electrons ("high resistivity") before exposure to light;
this is important for best performance. A set of thin conducting
electrodes of semitransparent "polysilicon" are applied.
Before exposure, the central electrode is set to a positive bias while
the two flanking electrodes are set negative. This creates a
"depletion" region under the central electrode containing no
holes but a deep potential well to trap electrons. The region shown
is about 10 µ thick.
OPERATION SEQUENCE: During exposure
(controlled by a separate shutter), light enters through the
"front-side" electrodes. Photoelectrons generated under the central
electrode will be attracted toward the electrode and held below it.
The corresponding holes will be swept away into the bulk silicon.
Good performance requires little diffusion of electrons away from the
potential well.
The surface of the CCD is covered with MOS capactitors in a pattern
like that at the right. In this particular design, there are three
electrodes per pixel. A single pixel is shown shaded in the diagram.
Typical pixel sizes are 10-40 µ. The "parallel
shift" registers are shown as rows running across the whole face
of the CCD. These are separated by insulating "channel stops."
At one end of the CCD is a column of "serial shift" electronics
and an output amplifier. There is only one amplifier in the design
shown. Contemporary large chip designs involve several amplifiers
(but always many fewer than the number of pixels!).
At the end of the exposure, readout of the collected electrons is
accomplished by cycling ("clocking") the voltages on the
electrodes such that the charge is shifted bodily along the
rows toward the serial shift column. The figure at the right shows
how this is done. Good performance depends on near-100%
transfer of the electrons to/from each electrode with no smearing
or generation of spurious electrons.
Each parallel transfer places the contents of one pixel from each row
into the serial register column. This column is then shifted out
vertically through the output amplifier and into computer memory
before the next parallel transfer occurs.
|
|
"BUCKET BRIGADE": The resulting
transfer and readout process is illustrated in the animation
below:
ADU CONVERSION: For storage in
memory, the electrical signal generated by the amplifier must be
digitized. This is done by an
"analog-to-digital converter". This is normally adjusted
such that one digital unit corresponds to
more than one
photo-electron. Typical values of this conversion are 2 to 8
electrons per stored digital unit.
The stored values are called
"ADU's", for
analog-to-digital-unit. The corresponding constant of transformation,
normally quoted in units of "electrons per ADU", is often called the
"Gain" (although this is confusing nomenclature because a larger Gain
results in reduced ADU values).
Note that the use of such a conversion importantly affects the
statistical properties of the recorded signal. If x is the
recorded signal in ADU's, y is the original signal in photo-electrons,
and G is the gain, then from
Lecture 8 we see that:
Var(x) = Var(y)/G2
VI. CCD DESIGN ISSUES
CCDs have undergone a long optimization process since 1980.
Contemporary designs have excellent performance but still require
careful calibration in order to overcome inherent limitations. There
is also only a handful of reliable manufacturers of
professional-grade chips.
Here are some of the issues affecting electronic design and
manufacture of CCDs:
- INTRINSICALLY LOW BLUE RESPONSE (< 4500 Å):
Caused by absorption in bulk Si and by electrode structures in
"Frontside-Illuminated" chips.
Mitigation:
- Use special thin, polysilicon material for electrodes; but these cannot
be completely transparent.
- Special Coatings: "Anti-reflection" coatings trap
photons, causing multiple reflections as in Fabry-Perot etalons, and therefore
enhance absorption. "Downconverter" coatings are phosphors which
absorb blue photons but emit green photons at wavelengths where the
CCD QE is higher (e.g. "mouse milk," coronene, lumogen).
- "Thinned, Backside-Illuminated" chips: shave off silicon
subtrate, leaving only a ~10 µ deep unit and illuminate from
backside; greatly improves blue response. The technique was pioneered
by Mike
Lesser at the University of Arizona.
Difficulties with thinned chips:
- Fragile
- Non-uniform thinning
- Surface trapping by SiO2 layer of photo-electrons produced
nearby (shorter wavelengths)
- Interference effects if wavelength ~ chip thickness (i.e. in IR).
Produce strong spatial modulation of response or "Fringing".
Especially serious for terrestrial night sky emission lines. Can be
well calibrated for narrow-band filters or for broad-band filters.
Harder for intermediate band filters.
- Thinning reduces red response. For good response
at 5000-10000 Å, thick (~500 µ)
front-illuminated chips are preferred.
- CHARGE TRANSFER EFFICIENCY (CTE)
- CTE can be better than 99.999% per transfer from one pixel to the
next, but it has to be, since the throughput of a chip with
2048 required shifts = CTE2048.
- It is possible to mitigate the effects of CTE reduction by revising
the technical design of a CCD (changing the number of phases or clocking
cycles; adding "minichannels") or operationally by adding a pedestal
background signal (see below).
VII. ADVANTAGES OF CCD DETECTORS
- HIGH QUANTUM EFFICIENCY: Reaching peak values of 80-90% in
the optical band. Much effort was expended to reach these high levels.
Sample response curves for three CCD's from ESO are shown below
right.
- This had tremendous impact on astronomical imaging &
spectroscopy. It meant the detection threshold with any instrument
was extended 4-5 magnitudes over film and that therefore a 1-m
telescope could now pursue the kind of science previously possible
only with 4-m class telescopes.
- A key corollary: since we are already near 100% QE, at least in
the optical region, achieving significantly lower threshold detections
requires larger telescopes rather than better detectors. This
was an important factor in the post-1980 rush to build ever larger
telescopes.
NB: "Quantum yield" can be over 100% for far-UV and X-ray photons
-- i.e. more than one photoelectron can be generated but
fewer than 100% of photons produce responses.
- LINEAR RESPONSE across the whole range of operation until an
exposure approaches full-well capacity (typically 200,000 e/pixel).
This allows much improved flux calibrations and much higher precision
for flux measurements at all levels than other 2-D systems.
- EXCELLENT DYNAMIC RANGE: Typically 104.
- WIDE WAVELENGTH RESPONSE: Intrinsically sensitive from
X-ray to ~1 µ. Arrays based on other materials
(e.g. InSb2, HgCdTe) with similar architectures are usable
in the IR.
- GEOMETRICALLY STABLE: excellent for astrometry
- ONLY LOW VOLTAGES REQUIRED (~5-15 v)
- RELATIVELY CHEAP, AVAILABLE, SIMPLE compared to other
digital 2-D systems. Standard chips cost ~$2-200 K.
- RELATIVELY LOW NOISE compared to many other classes of
astronomical detectors, e.g. Pg plates, Reticons, SEC vidicons, etc.
Dark current is largely suppressed by cooling. But noise is not
negligible. Typical equivalent read noise now is 2-10 e/pixel,
- SMALL PIXELS: typically 10-30 µ. Usually an advantage
in devising instrument/detector combinations, but designers should match pixel
size to 1/2 of smallest resolvable picture element in the optical
system.
- SPECIAL FORMAT/READOUT DESIGNS: By changing electrode structure
and clocking cycles, one can arrange for many different integrate/readout
modes.
Rapid clocking/video: inherent in CCDs intended for TV
applications and useful in some astronomical applications
(e.g. speckle interferometry). For bright sources, readout rates of
100 MHz are possible.
Drift Scanning:
The basic drift-scanning technique
(Schneider
et al. 1994) is to clock the chip slowly along its
parallel registers while moving the telescope to keep a target
centered on the moving electron cloud as it builds up. The chip is
continuously read out to produce a strip-image of the sky. The
integration time is set by the drift time across the chip.
The simplest approach is to align the CCD parallel registers
east-west, keep the telescope fixed on the sky, and clock the chip
westward at the sidereal rate. A sample application of drift scanning
is
described here.
Drift-scanning is now a standard method for wide-field CCD sky
surveys, most notably the Sloan Digital
Sky Survey
"Nod and Shuffle": this
technique takes advantage of the capability to shift an image
wholesale on the CCD without reading it out to obtain much better sky
subtraction (e.g. in the near-IR where atmospheric OH emission is very
bright and variable).
On-chip binning is possible by changing clocking to combine
electrons from several pixels together before reading out.
E.g. combine a 2x2 pixel region on the chip into a single
output pixel. This suppresses the effect of amplifier readout noise on
each pixel in the final data and also reduces memory and storage
requirements. It adds considerable practical flexibility to CCD
systems. Obviously, however, binning reduces the resolution of the
output image. Binning is useful for applications such as imagery of
very faint, extended sources (e.g. galaxy halos), low spatial
resolution spectroscopy, photometry of point sources under poor seeing
conditions, etc.
- UBIQUITY: CCDs and other solid-state array devices are now
almost universally used in astronomy (amateur & pro). Photographic
materials and older electronic detectors have been phased out, except
for specialized applications.
- IMMEDIATE DIGITAL CONVERSION OF DATA: The other advantages
of array detectors notwithstanding, it is the immediate conversion of
astronomical signals into a form capable of computer storage/analysis
that has so dramatically transformed UVOIR astronomy since
1980. The practice, pace, & scope of UVOIR astronomy are entirely
different now than in the "photographic" era that preceded widespread
use of CCDs and other array devices. Digital conversion of images and
spectra has enabled quantitative analysis of observations on a scale
not possible before.
Among other things, rapid digital processing allows near-real-time
assessment of data and hence much improved use of observing
time---notably in surveys (e.g. for variable sources in MACHO and
supernova searches; moving targets such as asteroids/Kuiper Belt
objects; or combined imaging/spectroscopic surveys such as SDSS,
2dF).
VIII. LIMITATIONS/DISADVANTAGES OF CCD'S
- SMALL SIZE: Individual chips typically are less than 7 cm
square. They cover only a small fraction of the high quality imaging
field on typical modern telescopes. Device size is limited by the small
fabrication yield of large, defect-free chips. The largest routinely
available chips are 4096x2048.
- However, mosaicking technology is now well developed. Typical
mosaics are now constructed from 4096x2048 chips.
- The largest CCD mosaic camera,
containing 201 CCD's, is being built for
the Large Synoptic Survey
Telescope
- Other examples: (click on images below for enlargements):
- Sloan Digital Sky Survey: 54 chip mosaic for drift scanning in 5 bands simultaneously
- Canada-France-Hawaii-Telescope MegaPrime mosaic: 40 4096x2048 chips covering
1 degree FOV
|
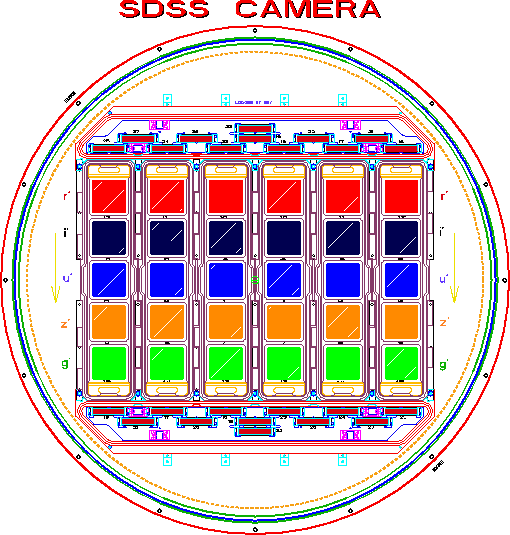 |
|
Sloan DSS Camera
|
Sloan DSS Camera
|
CFHT MegaPrime Mosaic
|
- CRYOGENIC COOLING REQUIRED: To reduce dark noise, cooling
to below -100 C is necessary. Thermoelectric coolers are usually not
adequate, so cryogens (e.g. liquid N2) are required.
Introduces many practical complications. Chips with excellent low-T
performance differ from commercial types used at room temperature in
digital cameras, cellphones, etc.
- READOUT NOISE: Produced by variations in amplifier gain.
There has been much effort to reduce readout noise, which is now
typically equivalent to 2-10 e/pix.
Even at these very low levels, RON can
compromise some types of observations (spectroscopy, surface
photometry), see Lecture 7.
What matters is not the noise per pixel but rather the total noise per
image area, which can extend over many pixels, depending
on the application.
RON effects in some applications can be reduced by using on-chip
binning (see above). In some cases, it may be more advantageous to
use "pulse counting" detectors, which can unambiguously detect
individual photons, rather than CCD's.
- FULL WELL CONSTRAINTS: Bright sources which over-fill
pixels can produce "blooming" or "bleeding" along columns, making
parts of the chip useless even far from the source. The best solution is
operational: e.g. by simply excluding overbright sources or by
breaking a long exposure into a series of shorter ones such that
saturation is avoided -- and then combining/stacking the result.
- RESPONSE NONUNIFORMITIES ("FLAT FIELD" EFFECTS): Caused by
small variations in masks used to manufacture chips, deposition
irregularities, thinning variations, etc. Typically 5%
pixel-to-pixel. Color-dependent. Requires extensive calibration,
with color-matching to targets. Use special exposures ("dome flats"
or "twilight flats"). Special observing procedures to suppress flat
field effects include:
- Drift Scanning: see above.
- Multiple Offset: Break exposure into 4-5 parts, offset ~50
pixels between exposures. Combine exposures during data reduction.
"Dithering" is a related technique involving smaller offsets to
achieve sub-pixel spatial resolution (see below). These methods
result in reduced field of view because not all parts of the original
field will have uniform exposures.
- FRINGING: see above. Caused by interference effects within
chip.
- SENSITIVITY TO COSMIC RAYS: High energy particles produce
strong, localized signals in CCDs, especially in thick chips. Their
effects must be removed during processing. CR's are a major
problem for spacecraft detectors (e.g. on HST). Mitigation
requires that exposures be broken into multiple parts (called
"CRSPLITs" on HST) so that CR events can be identified. CR hits can be
removed from processed data frames, but this always leaves "holes"
which have less exposure than other parts of the image.
- SENSITIVITY TO X-RAYS: An advantage for X-ray astronomy,
but X-rays from some materials in the vicinity of CCD's, e.g. special
glasses used for windows, can produce a diffuse background
which adds noise to observations.
|
CCD Flatfield Frame (AAO)
CR's on HST/WFPC2 2400 sec exposure
|
- CHARGE TRANSFER EFFICIENCY: Because of "traps" within the
CCD's, good CTE is often possible only for signal levels above a
threshold of ~10-50 e/pix. For good efficiency at low light levels,
this requires adding a "fat zero" (typically 1000 e/pix)
electronically or by "preflash" from a diffuse light source.
Unfortunately, this also creates added noise (10-30 e/pix). Long-term
exposure of CCD's to radiation in space on missions like HST
systematically increases the trap density, meaning that CTE reduction
is a
serious problem there.
- AVAILABILITY HOSTAGE TO COMMERCIAL MARKET: CCD availability
has always been driven more by commercial and military applications
than science. Scientific CCD manufacture represents only a few
percent of the overall $18B array detector market. A serious issue,
since
"active pixel sensors" (e.g. CMOS devices), a different
technology with amplifiers incorporated in each pixel, now dominate
the larger imaging array industry. Requirements for good performance
at low (astronomical) light levels are considerably different than for
room-temperature, short-exposure, mass-produced equipment.
- DATA GLUT! CCDs typically produce 2 x Npix
bytes of data per readout, where Npix is the number of
pixels. For a 8096x8096 mosaic, this is 130 MB. Data
storage/manipulation was a serious problem when CCD's were first
introduced, and this influenced the style of data processing systems
such as IRAF. Disk and solid-state storage are now much improved, but
long-term stability of
the massive
amounts of data now being produced by array detectors is a
non-trivial issue.
Cf. the LSST.
IX. EXAMPLE CCD SYSTEMS
- HST WIDE FIELD PLANETARY CAMERA 2 (1992)
- 4 CCD's, optically mosaicked with beam-splitter
- Loral 800x800, 15 µ pixel chips
- Front-illuminated, lumogen phosphor coating for UV response
- RON 5 e/px; QE (6000 Å) 35%
- SLOAN DIGITAL SKY SURVEY (1997)
- Drift scanning mosaic (not contiguous) containing 54 chips
- Tektronix/SITe 2048x2048, 24 µ pixel chips in 5x6
array; for simultaneous broad-band photometry. Both frontside and
thinned backside with AR coating. QE (6000 Å) ~60%; RON < 5
e/px
- Tektronix/SITe 2048x400, 24 µ pixel chips; for
astrometry & focus check. Frontside illuminated, RON < 15 e/px.
- HST WIDE FIELD CAMERA 3 (2001)
- 2 CCD's, physically mosaicked, contiguous
- Marconi 4096x2048, 15 µ pixel chips
- Thinned, back-illuminated; anti-reflection coating for enhanced blue/UV
response
- Minichannel for improved CTE
- RON 3 e/px; QE (6000 Å) 70%
- FAN
MOUNTAIN CCD SYSTEM (Gen II)
X. CCD HIGH PRECISION CALIBRATION PROCEDURE
A. DATA REQUIRED
- Bias frames
- Dark frames (optional)
- Flat field frames
- Sky flat/fringe frames (optional)
- Flux calibrator fields
- Target frames
B. REDUCTION AND DATA-TAKING PROCEDURES
- SUBTRACT BIAS FRAME: Bias frames (zero exposure time) are
taken with the chip unexposed to light. These measure the electronic
pedestal of the chip. For high precision, average many bias frames,
before and after observations. Check for bias drift during the night.
With some chips, you can determine the electronic bias level from the
"overscan" region on the chip.
- Optional: SUBTRACT DARK FRAME: Median filter many long (~30
min) dark exposures; note possible LED activity of CCD electrodes.
Scale result to integration time of each data frame before subtracting.
- DIVIDE BY TWILIGHT OR DOME FLAT FIELD: This removes the
residual pixel-to-pixel variations (typical 5%). Make exposures of
the twilight sky (good diffuse source, but tricky to get exposure level
right; only 2 chances per night). Or make many exposures of diffusely
illuminated screen in dome (disadvantage: often not uniformly
illuminated). Must be done for each filter used because of
color sensitive effects.
[Note: photon statistics must yield a SNR significantly in excess of
the final desired SNR -- i.e. if you want SNR = 100, the net
flat field image must contain well over 10,000
photons per pixel.]
- Optional: SUBTRACT SKY FLAT/FRINGE FRAME: Remove night sky
emission line fringing effects (worse in near-IR) by observing an
uncrowded field in the night sky. Take several exposures, moving
telescope by, say, 25 arc-sec between them. Use the "adaptive modal
filter" technique to zap star images and create a mean sky frame.
Scale to target frames and subtract. Resulting flat field as good as
0.1% can result. A sky flat determined this way is needed for each
filter.
- TARGET FRAMES: USE MULTIPLE OFFSETS, DITHERING OR DRIFT
SCANNING: For faintest possible photometry, use the
multiple-offset exposure technique -- e.g. 500 sec exposures shifted by
about 20 arc-sec each -- to reduce flat field errors and
identify cosmic rays.
You always want more than one exposure anyway for "reality
checks" and empirical determination of photometric errors and
transient effects.
- REGISTER AND COMBINE TARGET FRAMES: Re-register the offset
frames to sub-pixel accuracy (using, e.g., "Drizzle" softare),
creating an image "stack."
- REMOVE COSMIC RAY EVENTS: Median filter the signals in each
pixel in the stack. Outlying signals caused by cosmic rays and
other transients are thereby rejected from the combined image. You
must track the remaining "good" exposure time for each pixel.
- COSMETIC TREATMENT: trim off the "lost" parts of the
image. Depending on the application, you can interpolate over or mask
out bad pixels.
- CALIBRATE FLUX SCALE: Observe "standard stars" in
recommended CCD calibrator fields (e.g. star clusters) to determine
nightly atmospheric extinction and telescope throughput. No need to
take on non-photometric nights (e.g. variable clouds), but no flux
calibration for those, either. Calibrator stars should overlap
targets of interest in color. These frames are reduced exactly like
target frames, apparent fluxes extracted, and the multiplicative
factors needed to convert to true fluxes of targets are obtained.
- APPLY GOOD PHOTOMETRIC REDUCTION SOFTWARE: for source IDs,
flux measurements (point or extended source).
=====> RESULT : PHOTOMETRIC CALIBRATION
GOOD TO 0.005 MAG
XI. EXAMPLE SCIENTIFIC APPLICATIONS OF HIGH PERFORMANCE CCDs
- Deep imaging using subpixel registration. This example
employs dithered HST imaging and A. Fruchter's "drizzling" technique.
See the image pair below. On the left is one original frame (I-band, 2400
sec exposure). Most of the "features" in this image are cosmic ray
tracks, not cosmic objects. On the right is the result of drizzled
processing of 12 such frames. The combined image has a limiting
magnitude of I ~ 28. It would be impossible to reach such levels with
photographic plates or other less-stable and calibrateable systems..
- Color-magnitude diagrams. An example of the improvement
offered by CCD's over photographic photometry in a CMD for the
globular star cluster 47 Tuc is shown below. On the left is a 1977
state-of-the-art CMD based on widefield photographic images with
photoelectric calibrations (Hesser & Hartwick, 1977). On the right is
a 1987 CMD derived from 4-m CCD photometry (Hesser et al. 1987). The
greatly improved photometric precision reveals new features of
astrophysical interest: e.g. the thinness of the main sequence near
turnoff, which places strong limits on the range of ages present in
the cluster, and the shape of the subgiant and asymptotic giant branches.
(Later, yet higher precision, CCD imaging with HST in the
near-ultraviolet bands revealed the existence of multiple populations
with similar ages but different metal and He abundances in many
globular clusters.)
Photographic CMD
CCD CMD
|
|
- High precision stellar photometry with Kepler. The Kepler
spacecraft searched for exoplanet transits of 10-16 magnitude stars
using a 42-chip CCD mosaic camera. To reach exoplanets of Earth size,
the transit method requires extremely high precision photometry, which
is possible in space given the absence of atmospheric interference.
Kepler reached a photometric precision of ~30 parts per million at
12th magnitude --- the best ever achieved in astronomy to that time.
A sample light curve is shown below (note the flux scale):
- Multi-object spectroscopy. CCD's are widely used as
detectors in spectrographs. They were the basis for the flourishing
of multi-object spectroscopy, which now extends to the simultaneous
observation of hundreds of sources in a given field. Examples of
these powerful systems are described
in Lecture 10. A sample stack of 46,000
QSO spectra from the first Sloan Digital Sky Survey is shown below.
It would be wholly infeasible to collect such large samples of
astronomical spectra without the powerful capabilities of area
detectors like CCDs.
- High precision astrometry with Gaia. The Gaia space
astrometry mission employs a focal plane with 106 specially-designed
CCDs fed by two telescopes with different lines of sight and operated
in drift-scan mode. To reach its intended limit of ~20 mag, it uses
the full 3300-10500 Å band available to the CCDs. It reads data
only for selected targets in each field, and each of these is
identified by on-board software from a special set of pre-scan mapping
CCDs. Gaia achieves an unprecedented precision of about 30
micro-arc-seconds for parallaxes of stars of 15th magnitude.
XII. NON-UVOIR USE OF CCD & RELATED DEVICES
A. X-RAY: CHANDRA/ACIS DETECTOR
ACIS is a 10-CCD (1024x1024 chips)
focal plane array used on Chandra for both imaging
and spectroscopy. It uses both back-illuminated and front-illuminated
versions.
It is operated in a pulse-detection mode, unlike the standard
procedure at UVOIR wavelengths.
Each X-ray photon releases more than one electron in the
CCD, in fact, the mean number released is ~ EXR/3.7 eV.
Since Chandra operates at ~ 5 keV, the average electron cloud
corresponding to one photon has ~ 1000 electrons.
The standard operation sequence is to expose for 3.2 seconds, then
rapidly read out the array in 40 ms. The resulting image is
analyzed by on-board software to catalogue the x,y position and
the pulse amplitude of each valid photon pulse.
Because the pulse amplitude is proportional to the photon energy, ACIS
offers an intrinsic spectral resolution of R ~ 10-50.
A difficulty with the ACIS design is that if more than one photon
strikes the same pixel during the exposure time, the counting
analysis becomes distorted, and the photon flux is underestimated.
This is called "pileup." Fortunately, most X-ray sources are faint
enough that this is not a problem.
B. INFRARED ARRAYS
The table in Sec. IV above shows that pure silicon
photoconductor arrays will not work at infrared wavelengths because of
their large band-gaps, but there are a number of other materials that
will.
There are many varieties of IR detectors in use today. Some of
these are monolithic, i.e. fabricated on single subtrates like
CCD's, and some are
hybrids in which the readout electronics
are fabricated separately from the photon-detection devices.
Hybrids typically use silicon wafers for the readout electronics.
Some actually use CCD-type architecture. The readout is connected to
the photon-sensing material using conducting "bump-bonded" indium
studs. If the wafers ultimately produce readout though a small number
of output amplifiers, they are called "multiplexers" or "MUX's".
Here is
a cross-section of the HST/NICMOS infrared detector, which uses
a HgCdTe ("mercad-telluride") photon detector.
ADDITIONAL WEB LINKS
CCD-World
(forum for discussions about CCDs and their use in astronomy)
Basics:
Example Systems & Development Efforts:
Last modified
April 2023 by rwo
Bandgap images from Britney's Guide to Semiconductors.
MOS capacitor illustration from Molecular Expressions. Bucket brigade
animation and front/back illumination drawing by C. Tremonti (UWisc).
CCD design drawings from C. MacKay, Annual Reviews (1986). Most other
images from public observatory sites. Text copyright © 1989-2023
Robert W. O'Connell. All rights reserved. These notes are intended
for the private, noncommercial use of students enrolled in Astronomy
511 at the University of Virginia.