ASTR 1210 (O'CONNELL) SUPPLEMENTS
There are three special supplements which are required reading for
this class.
Supplement I (a PDF
file) contains reference material on units of measurement, powers of
ten, nomenclature, and special symbols. It should be
consulted as
necessary throughout the remainder of the course.
Supplements II and III are intended
to
replace the material in the textbook covering the basic
properties of matter, the nature of forces, the electromagnetic
spectrum, and how astronomers exploit the properties of the EM
spectrum to deduce the physical nature of distant objects. They also
serve as the
Study Guides for the
lectures where this material is covered. Most of the concepts
presented in II and III should already be familiar to you. These
supplements are available in this HTML file.
ASTRONOMY 1210: SUPPLEMENT II
A. Elementary Particles
You should already be familiar with three elementary particles:
the
electron, the
proton, and the
neutron.
These are the primary constituents of
atoms. Atoms were first
proposed as the basic components of matter by Greek philosophers (e.g.
Democritus, ca. 400 BC), and their reality was debated for thousands
of years. Their existence was proven beyond doubt in the 19th
century.
Protons and neutrons are bound together in the
nucleus of the atom, an
object about 10
-13 centimeters in diameter. The electrons
orbit the nucleus in a cloud which is 100,000 times larger,
10
-8 cm in diameter. There is a multitude of other
elementary particles, most of which exist only for very short periods
of time; most of these will not be discussed further in the course.
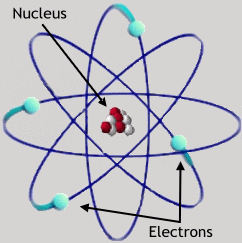
Two properties of elementary particles are relevant for us: their
mass and
charge.
Roughly speaking,
mass is the "amount of matter" in an object.
Quantitatively, it is the
inertial mass and measures the
"resistance" of an object to an applied force (as expressed in Newton's
Second Law). The larger the mass, the harder it is to move the object.
Mass is measured in grams:
- Mass of proton (or neutron) = 1.7 x 10-24 grams
- Mass of electron = mass of proton/1800
Charge is a property of particles which determines their behavior
when acted on by
electromagnetic forces . "EM" forces are described
in more detail in section B.
All elementary particles which have been isolated are found to have only
certain definite amounts of charge: none, exactly one positive unit
of charge, or exactly one negative unit. (Protons and neutrons
are constructed of even more basic subunits
called "quarks", which have fractional charges. But these are strongly
bound together inside protons and neutrons. Free quarks
should rarely exist and have never been observed in the laboratory.)
- The neutron has zero charge
- The proton has a charge of +1
- The electron has a charge of -1
(Note: The + or - sign is simply a convention
indicating that electrons and protons behave differently in EM force
fields.)
Since the nucleus of an atom contains only protons and neutrons, it will have
a positive charge. The basic property that distinguishes the various
elements from one another is the
number of protons in their
nuclei or, equivalently, the amount of positive charge on the nucleus.
There are
92 naturally occurring elements on the earth, ranging from
hydrogen (with only one proton in its nucleus and no neutrons) to
uranium (with 92 protons and 146 neutrons). [Other elements, e.g.
plutonium, have been artificially produced, but all of these are
unstable and have finite lifetimes.]
All materials, despite
their myriad appearances, are made of these 92 types of atoms. In
fact, most everyday materials, including living things, are made of no
more than
about 20 types of atoms.
Note: the abundances of elements on the Earth are very different from
their cosmic abundances (i.e. those in the stars or the
interstellar gas). 75% of the earth's crust is oxygen or
silicon. However, in our galaxy, hydrogen makes up about 72% of the
total mass, helium another 25%, and all other elements only 3%.
In their undisturbed or "neutral" state, atoms have as many electrons
as protons and therefore have a
net charge of
zero. Under many
circumstances, however, electrons can be stripped out of the cloud
which surrounds the nucleus. The atom is then said to
be
ionized and is referred to as a "positive ion" since it has
a net positive charge.
The last elementary particle we will discuss is the
photon,
which is not a "regular" particle at all. It has zero mass
and zero charge. Instead, the
photon is a packet of electromagnetic
energy. The concept of the photon is a product of the
quantum
theory, which describes the behavior of elementary particles. The
photon is the "particle" which transmits the electromagnetic force
between charged particles. Light waves consist of photons, as do radio
waves, and the discrete, particle-like impact of photons on special
detectors can actually be readily demonstrated in the
laboratory. Photons are discussed further in Supplement III.
B. Forces
If particles did not exert forces on each other, there would be no
organization in the universe: no planets, no stars, no people.
Particles would move throughout space in random but unchanging paths,
never altering their motion even when they passed through one
another.
However, particles do exert forces on each other, and the
nature of these forces depends on the mass, charge (and other
"internal" properties we will not discuss), and state of motion of the
particle.
Isaac
Newton was the first to formulate a quantitative
description of
how matter responds to forces which are
applied to it. Historically, Newton's laws were a major stimulus
for both the scientific revolution and the industrial revolution since
they demonstrated that the universe is understandable through rational
analysis. His description is encapsulated in his
second law of
motion :
where F is the force applied to an object, m is the
object's inertial mass, and a is the object's
acceleration.
Newton's formulation is now known
not to apply on microscopic
scales, such as to sub-atomic particles. On macroscopic
scales, however, it is an excellent approximation and is the basis,
for example, of structural, mechanical, and aeronautical engineering.
Acceleration is the
rate of change of velocity. An object is
said to accelerate if
either its speed or
direction of motion changes. It is the only one of the three
parameters in the second law (force, mass and acceleration) which can
be readily observed. So, it is useful to solve the equation for
acceleration:
This expression implies that for a given mass, the larger the force
the larger the acceleration. Also, for a given force, the larger the
mass the smaller the acceleration. A boxcar is more massive than your
little brother and is therefore harder to push around.
As the rate of
change of a quantity (velocity), acceleration is the mathematical
derivative of velocity (which is itself the derivative of
position). Also, the force may depend on position, velocity, and
other properties of the system. Thus, this expression is actually
not a simple algebraic expression but instead is a differential
equation. In order to solve it, Newton was forced to develop
calculus, one of the most powerful and valuable intellectual
tools ever invented.
Newton recognized only two kinds of force: (i) gravity, and (ii) the
force produced by physical contact (e.g. you pull on something, a
spring pushes something, the wind shifts something, etc.). We now
realize that all varieties of this "physical contact" force are
manifestations of
electromagnetic force. But this was not
understood until over 150 years after Newton published his Second Law.
Fortunately, it was not necessary to know how objects
produced
the forces they exerted on one another in order to predict how they
would
move in response to those forces.
There are
three categories of forces exerted by particles on
each other that are now recognized: gravity, nuclear forces, and
electromagnetic forces. Forces can be characterized by their
strength, their
range, and their
coupling
(that is, which properties of particles determine the response to a
particular force).
Gravity
The gravitational force is an
attractive force between any two
objects containing
mass. All particles in the universe attract
each other; any pair of particles which start from rest will
eventually move together (in the absence of other nearby particles).
Gravity seems strong and inexorable in everyday life, but in fact it
is by far the
weakest of the known forces. Gravity falls off
with distance, but is a
long-range force compared to the
nuclear forces (e.g. it can act over intergalactic distances).
Newton was the first to develop a quantitative theory of gravity. In
Newton's formulation, gravitational force acts along the line
connecting the two objects and is proportional to the
product of the masses of the two objects but inversely proportional to
the distance between the two squared. That is,
where G is a numerical constant, M1 and M2 are
the masses of the two objects, and R is the distance between the two.
Newton showed that, when combined with his second law, this force law
correctly predicted the observed motions and orbits of the planets.
In particular, the predictions were in complete agreement
with Kepler's Laws of planetary motion, which had been derived
from the extensive observations of Tycho. See
Study Guide 8 for more details.
Newton's theory was shown to be inadequate by Albert
Einstein
(1916) in the presence of large masses or over large distances, and
has been replaced by the General Theory of Relativity in such
situations. Relativity theory profoundly changed our understanding of
space and time. But as a practical matter, Newton's theory is an
entirely satisfactory description of "everyday" gravity. Only very
minor corrections to the Newtonian predictions are necessary, for
example, to send spacecraft with high accuracy throughout the solar
system.
Nuclear Force
This is a
short range force, acting
over distances of only 10
-13 cm (i.e. about the size of an
atomic nucleus). It has no direct influence over significantly larger
distances. (The longer range effects of nuclear materials, e.g. in
radiation therapy, are produced by particles or photons ejected from
atomic nuclei by the nuclear forces, not the forces themselves.)
Because this is the case, the effects of nuclear force are described
by the
quantum theory and not Newton's Second Law. Technically,
nuclear force is subdivided into the
strong and
weak forces (and hence you will often find references to the
"four forces" of nature, rather than the three described here).
Inside the nucleus, nuclear forces are very strong, some
1040 times stronger than gravity(!), which is wholly
negligible in nuclei. The nuclear forces bind protons and neutrons
together in nuclei. They are responsible for nuclear power,
radioactivity, and the explosive energy of nuclear bombs.
[Note that "atomic bomb" is really a misnomer, since it is not atoms
that release the energy in a fission or fusion bomb but only their
nuclei. Chemical explosives (e.g. dynamite) are the real
"atomic bombs" because their energy release originates from changes in
the electron shells around the nuclei.]
Electromagnetic Force
The ubiquity of the effects of
electromagnetic (EM) force make it the
most important in
everyday life. It is, of course, responsible for electricity
and magnetism. But it is also the source of combustion, solidity,
friction, capillary action, etc. In short, it holds our everyday world
together. EM force is responsible for all
chemical reactions,
including those occurring in biological organisms.
The simplest form of EM force is the electrostatic force ,
which acts between any two objects with net charge which are at
rest. If the two charges have the same sign (both + or both -), the
force is repulsive and the objects tend to move apart. If the two
charges are opposite, the force is attractive (like gravity). The ES
force is a strong force: the ES repulsion between two electrons
is 1036 times stronger than the corresponding gravitational
attraction. It is also in principle a long range force--but
not in practice, since macroscopic objects tend to have zero net
charge.
The magnetic force is more complicated. It acts in a complex
manner on moving charged particles. Two objects with zero net
charge can interact magnetically if charges inside of them move in
sufficiently organized patterns ("currents"). A bar magnet is an
example of an object with zero net charge which can be affected by
external magnetic fields. The Sun, the Earth, and most other planets
also have fairly strong magnetic fields, which affect the motion of
particles near them. On the Earth's surface the most familiar
magnetic effect is found in the orientation of a compass needle to the
Earth's "magnetic poles". Magnetic forces are also long
range but are much more important on galactic scales than are
electrostatic forces because they are usually not self-shielding like
ES forces. On such scales they are usually less important than
gravity, however.
Notes:
We have never been able to identify any special interaction (e.g. a
"life force") that is harbored only by living beings and governs their
functioning, behavior, or mental processes. EM forces control all biological
phenomena, including
consciousness.
EM force is the only kind of interaction which could be fashioned into
the mysterious "force fields" favored by science fiction writers or
could be responsible for claimed borderline phenomena like
telekinesis, extra-sensory channels of communcation, etc.
Atoms contain a nucleus with positive charges
and a surrounding electron cloud of negative charges. It is
the
EM forces between these charged particles that hold atoms
together. Michael
Faraday's (ca. 1830) investigations of the
electrical properties of materials provided the first clues to this
fundamental discovery, and incidentally made it possible to utilize
electrical power as the basic tool of modern civilization (see
Study Guide 9). Nuclear
forces do not play a major role on the scales corresponding to the
sizes of atoms; they are important only inside the nucleus.
When two atoms approach each other, their constituents feel a
combination of attractive and repulsive forces, depending on the exact
configuration of the nuclei and electrons. Under the right
circumstances, atoms can bond together---i.e. the attractive
force overcomes the repulsive force---and create a stable system.
This is called a molecule. Most molecules are relatively
simple, consisting of only a few atoms (water consists of only three),
but organic molecules can be of enormous size, containing thousands to
millions of individual atoms.
Chemical energy is released when the electronic configuration
of molecules is altered through interactions between them.
Fire is perhaps the most obvious everyday example of chemical
energy release.
Atoms in a crystal
Solids
are formed when atoms and molecules are in a sufficiently dense
material that their electron clouds interpenetrate and form a bond
strong enough to keep their nuclei in an approximately rigid
configuration. In some solids, electrons are held tightly to their
nuclei. In others, electrons are free to move in response to external
electromagnetic fields; these materials are called conductors of
electricity.
ASTRONOMY 1210: SUPPLEMENT III
A. Electromagnetic Waves
Based on the experimental studies of Faraday and others, especially
Faraday's discovery of electromagnetic induction and his concept of a
magnetic force field, James Clerk
Maxwell managed to summarize
(ca. 1865) all electromagnetic phenomena in a set of elegant and
interconnected equations now known as
Maxwell's equations.
These represent the
third great generalized physical law, the
first two being Newton's equations of motion and his theory of
gravity.
Maxwell was able to show that his equations implied that
electric and
magnetic force fields can
propagate through space.
A moving electron, for example,
sends out a disturbance which can cause other electrons to move;
"target" electrons at successively greater distances will feel the
disturbance at successively later times. The propagating disturbance
is similar to a water wave produced by a rock dropped into a pond and
is therefore called an
electromagnetic wave.
One of Maxwell's great discoveries was that he could use his equations
to
predict the velocity of an EM wave as it propagated through
a vacuum. He found the velocity to be the same as the
speed of
light, which had already been determined with fair precision. The
immediate inference was that
light is one kind of
electromagnetic wave.
Maxwell's theoretical prediction of EM waves was later
confirmed experimentally by Heinrich
Hertz (1887), who detected, for
the first time, artificially generated
radio waves. Only a few years
later in 1895, the physicist Wilhelm
Röntgen discovered high energy
EM waves in the form of
X-rays and simultaneously recognized their
tremendous penetrating power and usefulness as medical diagnostics. Maxwell's
prediction of EM waves has had profound practical consequences.
Wave disturbances
EM waves are classified by their wavelength or
frequency. By analogy to water waves, the wavelength of an EM
wave is the distance between crests (or places where the EM effects are
strongest). See the figure above. The frequency is the number of
crests passing the observer per second. If the waves move with a
definite velocity, then it is easy to see that wavelength and
frequency must be inversely related to each other. The longer the
wavelength, the lower the frequency; and the shorter the wavelength,
the higher the frequency. Wavelength is measured in whatever unit of
length is most convenient: from centimeters or millimeters at long
wavelengths to Ångstroms (where 1 Å = 10-8 cm)
for optical light. Frequency is measured in cycles ("crests") per
second or Hertz (1 Hz = 1 cps).
B. The Electromagnetic Spectrum
All frequencies from zero to infinity are possible for EM waves, and
this total range is called the electromagnetic spectrum. See
the figure below; the textbook presents other illustrations and more
information. Radio, television, microwaves, infrared, visible
light, ultraviolet light, X-rays, and gamma rays are all forms of EM
waves. All of these waves travel at the same speed (at
least in a vacuum): the speed of light, which is 300,000 kilometers
per second (186,000 miles per second).
Full EM spectrum with visible spectrum enlarged
(Units marked
are microns. 1 micron = 10,000 Å)
The human eye is directly sensitive only to a very small range of
wavelengths in the EM spectrum. This is called the "visible" or
"optical" region. It extends roughly from wavelengths of 4000
Å in the deep violet to 7000 Å in the deep red. Green light has
a wavelength around 5000 Å, or about 0.0005 mm.
The wavelengths or visible light are far smaller than
sizes encountered in everyday life. Therefore,
we are not conscious of light's wavelike character.
However, radio waves are long enough that wave effects such as
diffraction are obvious: the fact that radio waves can reach
you even if the transmitter is hidden behind a building is a result of
the waves diffracting around the building, just as water waves can
move around a rock near the beach. (For more on diffraction, see
Study Guide 14.)
Applet. Here
is an interactive Java applet illustrating wave diffraction.
Although our eyes are not sensitive to most EM wavelengths, sensors in
our skin can detect
infrared EM waves (10,000-100,000 Å), which
we perceive as
radiant heat. Shorter wavelengths in the
ultraviolet and X-ray cannot be sensed directly by our bodies;
but these can cause damage to cells. For instance, damage resulting
from exposure to ultraviolet light appears as "sunburn".
Fortunately,
most of this harmful cosmic X-ray and UV is screened out by the Earth's
atmosphere and does not reach sea level.
In fact, the
Earth's atmosphere is opaque to most wavelengths
in the EM spectrum. The chart below shows the ability of different
wavelengths to penetrate the atmosphere. The screening is protective
for lifeforms on Earth's surface. But obviously, it is not convenient
for astronomers who want to monitor the universe across the full EM
spectrum. (This is the main motivation for
space astronomy.)
The atmosphere also absorbs large parts of the Earth's infrared
spectrum, which leads to the atmospheric heating phenomenon known as
the
"greenhouse effect" (see
Study
Guides 15 and
19.)
Penetration depth in Earth's atmosphere
for
different kinds of EM waves
Notes:
Sound is not an EM wave. A sound wave is a
compressional disturbance in a medium (air, water, etc.) and
propagates far more slowly than EM waves. Sound waves cannot
exist in a vacuum, whereas EM waves can.
The difference in propagation speed between sound and light is
vividly apparent during a thunderstorm. The light of a lightning
flash will reach you almost instantaneously, whereas the sound travels
at a speed of only 1100 feet per second, so there will be an
appreciable lapse between the flash and the sound of the corresponding
thunder. To estimate the distance of the lightning flash (in feet),
simply multiply the number of seconds between the flash and the sound
arrival time by 1100. A 5-second delay means the lightning strike
was over a mile away.
Also, most "atomic or nuclear radiation" is not electromagnetic
either. Instead, it consists largely of highly energetic subatomic
particles. It is dangerous to living things because of its
potential to ionize biological molecules, including those in
genes.
EM waves are also often referred to as "EM radiation." But it
is important to distinguish long-wavelength EM waves (e.g.
from a computer terminal or microwave oven), which are
almost never dangerous in everyday situations, from more threatening
kinds of "radiation."
C. Photons
Although EM radiation does have many wavelike properties, it is
not simply a wavelike disturbance. It is absorbed or
emitted from particles in tiny packets, called
photons , which
themselves behave more like particles than waves. The energy of a
photon depends on its frequency (in the wave analogy): the higher the
frequency, the larger the photon's energy.
Photons are created or destroyed by atoms through interactions
with the charged particles within the atom. According to the
quantum theory, the internal energy states of an atom are
discrete or quantized. This means that the electron cloud
around the nucleus of the atom can exist only in certain definite
energy states. Whenever the cloud changes its energy state, a certain
definite amount of energy must be lost (if the energy drops) or gained
(if the energy increases). One of the most important ways in which
atoms lose or gain energy is through photons. The electron cloud can
spontaneously lose energy by emitting a photon,
which then escapes from the atom. Alternatively, it can
absorb
a photon which is near the atom, thereby gaining energy (and
destroying the photon).
D. Spectroscopy
As a consequence of sponteneous photon creation by atoms, all
objects which are above a temperature of absolute zero emit
electromagnetic waves---even textbooks and ice cubes. There is a
great deal of information about the physical properties of the object
contained in the distribution of wave energy as a function of
wavelength or frequency in its electromagnetic spectrum. For short,
this distribution is called the object's spectrum.
"Spectroscopy" is the study of EM spectra.
The spectrum of a dense object like the Earth or Lake
Superior or the inner regions of the Sun is smooth or
"continuous" and changes only slowly with wavelength. You can
think of the atoms and electrons in such an object as interacting so
strongly that the individual signatures of each type of atom are
blended away. Such an object is said to be in thermal
equilibrium, and the resulting spectrum is sensitive only to
its temperature. This kind of spectrum is called a blackbody
spectrum, named after ideal, laboratory sources which are purely
self-luminous and reflect no light. Such sources have a spectrum with
a smooth, well-defined, characteristic shape, as shown in the figure
below:
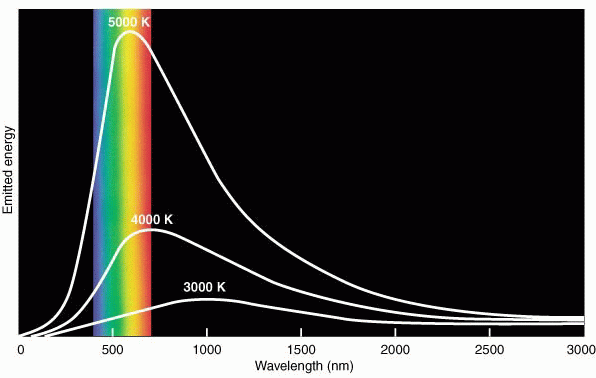
This diagram shows how temperature affects the
distribution of the emitted blackbody spectrum of a dense object.
(The wavelength scale is in nanometers. 500 nm on this scale
corresponds to 5000 Å or 0.5 microns.) The colored bands show
the "visible" part of the EM spectrum.
As shown here, hotter objects emit more energy at
shorter wavelengths while cooler objects emit more energy at
longer wavelengths. Hence, hot stars will look blue-white to the eye
while cool stars look orange-red. The spectrum of the Sun, with a
surface temperature of almost 6000o Kelvin peaks at about 5000
Å --- i.e. in yellow-green light.
Does this fact, combined with the principle of natural biological
selection, suggest a reason why our eyes are most sensitive to
yellow-green light?
An incandescent light bulb operates by using an electric current to
heat a thin metal filament until it glows brightly, at about
2500o Kelvin. Higher temperatures would vaporize the
filament. Hence, incandescent light bulbs are redder than the Sun
and, in fact, redder than any of the stars visible to the unaided eye,
which all have temperatures above 3000o Kelvin.
On the other hand, the Earth, with an overall mean surface temperature
of only 287o Kelvin (14o C), peaks at a
wavelength of about 100,000 Å (10 microns) in
the infrared part of the spectrum. Since our eyes are not
sensitive at this wavelength, the Earth looks "dark".
Objects at room temperature (about 300o Kelvin) also
emit their peak radiation in the infrared. They
are self-luminous at those wavelengths, but the light we can
detect from them with our eyes is not their own self-radiation
but rather
reflected light from higher temperature sources (such as the
Sun or incandescent light bulbs).
For instance, see the image below, which compares the visible-band
(reflected light) with the "thermal-infrared" appearance of a cat
(with a body temperature of about 312 Kelvin).
The total radiative output from a blackbody source (summed over
all wavelengths) is a very strong function of its temperature and
scales as the temperature (on the Kelvin scale) to the fourth power
(T4).
Here is a Java demo of the effects of temperature on EM
radiation.
So far, we have discussed dense systems. By contrast, in a dilute or
thin gas---the outer atmosphere of the Sun or an interstellar
gas cloud, for example---the atoms do not interact strongly, and if
there is a large enough number of atoms of a given type (e.g. hydrogen
or oxygen), they will impress their
individual signatures on the emergent spectrum.
If you could pump up a single atom to a high energy state and then
observe it sitting quietly without further outside disturbance, you
would find that it would emit a series of photons with discrete, but
different, energies as it lost energy from its electron cloud. Thus,
its spectrum would consist of a series of sharp peaks: only certain
frequencies or wavelengths would be included. It would be faint (or
dark) except at the peaks. This is called an "emission line
spectrum" because if you plotted the number of emitted photons versus
their frequencies, it would resemble a picket fence.
If you imagine the same atom left in a low energy state but placed
in front of a dense, glowing object, you would observe a spectrum which
was the reverse of the atom's emission line spectrum. Here, you
would see light from the background glowing object at all frequencies
except where the object's photons could be absorbed by the electron
cloud. This is an "absorption line spectrum": dark at those
discrete frequencies where the
electron cloud can absorb energy but bright elsewhere.
The 3 general kinds of spectra are summarized in the illustration
below:
The essential practical implication of these facts stems from the fact
that the spectrum of each type of atom is different because its
electronic structure is different. A hydrogen atom has a
different spectrum than a helium atom, and carbon atoms have a yet
different spectrum. Obviously, then, the emission or absorption line
spectrum of a star or planet is related to its chemical
composition.
It is, in fact, through analysis of such spectra that we know the
chemical content of stars, the interstellar gas, and other galaxies
even though they are tremendously far away.
For instance, the figure below shows part of the spectrum of the Sun
at yellow wavelengths. The dark lines are produced by atoms of
magnesium, iron, titanium, and other elements in the cooler, outer
layers of the Sun which are seen against the bright continuum produced
by its hot inner regions. Quantitative analysis of such spectra tells
us the detailed chemical makeup of the Sun. For example, the
element helium (the second lightest after hydrogen) was
discovered in the spectrum of the Sun before it was studied in
an Earth-bound laboratory. Click on the image for an enlarged
spectrum of the Sun.
Here is a Java applet that
allows you to see the optical spectrum of any element in the periodic
table.
In addition, spectra also contain information on the velocity,
pressure or density, temperature, ionization state, magnetic field
strength, and other physical parameters of the material which forms
them.
Spectroscopy is therefore an exceedingly powerful tool. It is
the source of most of the physical information we have about
astronomical objects which are too distant to sample directly with
spacecraft (i.e. most of the universe).
Bad Philosophy Footnote: Click here for
a related description of one of the worst, but not one of the last,
faulty prognostications about science by a philosopher.
E. Luminosity and Flux
The luminosity of an object is defined to be the total
amount of electromagnetic energy radiated by the object in a given
time. It may refer to the whole EM spectrum, in which case it is
denoted a "bolometric" luminosity, or it may refer only to a limited
part of the spectrum. For example, the "visual" luminosity of a star
refers to the total energy output in the visible part of the
spectrum. It is denoted LV.
Luminosity is an intrinsic property of the object; that is, it
does not depend on how far away the observer is. "Intrinsic
brightness" or "power" are synonyms for luminosity. In the cgs system,
luminosity is measured in ergs per second.
The response produced by an object of given luminosity in a detector
(for example the human eye) depends both on the luminosity and the
distance of the object. More distant objects produce smaller
responses. The response of a detector to a given source of EM
radiation is a function of the flux of radiation from the
object. The flux is defined to be the energy crossing a given area
in a given time. It is measured in ergs per second per square cm
(ergs/sec cm2) in the cgs system. Flux is not an
intrinsic property of the source because it depends on the observer's
distance. The greater the flux, the greater the response of a given
instrument. Flux can be defined with respect to part or all of the EM
spectrum.
A little thought will lead you to realize that flux = (total energy
output of source per unit time)/(area of sphere passing through observer with center
on source). Or,
where F is the flux from the source, L is its luminosity, and R is its
distance from the observer. Like the expression for Newtonian
gravitational force, this is an "inverse square law".
F. The Doppler Effect
The Doppler Effect is a change in the apparent wavelength or
frequency of an EM wave caused by the motion of the source of the wave
toward or away from the observer. It also occurs with sound waves,
where it is most familiar as the change in pitch of a police or
ambulance siren as it passes you on the street. When the siren is
approaching you, the pitch is higher (i.e. the frequency is higher and
the wavelength is shorter) than when it is receding from you (lower
frequency, longer wavelength).
The Effect is explained by the fact that if a source is approaching you
while emitting a wave of fixed wavelength, each successive crest of
the wave starts from a position a bit closer to you than the preceding
one. But the crests still travel at the same velocity. This means
that the distance between successive crests (the wavelength) traveling
in your direction will be smaller than if the source were stationary.
The stream of crests moving in your direction is therefore more
"compressed" than for a source at rest. You will observe a signal
with shorter wavelength (higher frequency) than that from the same
source at rest. Conversely, if the source moves away from you, the
stream of crests is "stretched", and you will observe a longer
wavelength (lower frequency). "Doppler radar" makes use of this
phenomenon to measure the motion of air streams in the atmosphere or
of automobiles on the highway (often in the hands of the state
police).
Here
is a nice Java applet illustrating the Doppler Effect. You can
interactively adjust the velocity and direction of motion of the
source. Note how the propagating waves are compressed along
the direction of motion but expanded along the opposite direction.
The magnitude of the effect depends on the velocity of the source.
If the source moves faster than the speed of propagation in
the surrounding medium, a cone-like "shock wave" results; this is what
produces a "sonic boom" from a supersonic jet plane in the atmosphere.
However, since no source can move faster than the speed of light in a
vacuum, this case doesn't apply to light waves emitted by objects
moving in space.
Applied to astronomical spectra, the Doppler Effect is particularly
valuable, since it allows us to measure the motion of distant
objects toward us or away from us --- i.e. what astronomers call the
"radial velocity." By measuring the exact wavelengths of spectral
emission or absorption lines and comparing them to the expected values
for atoms which are at rest, we can estimate the motion of objects
along our line of sight (i.e. radially). The method does not depend
on the distance of the object. Since the change in wavelength is
toward the blue end of the spectrum if the object is approaching and
toward the red end if it is receding, astronomers speak of "blue
shifts" and "red shifts." The cosmic red shift, observed
in the spectra of distant galaxies, is the principal evidence that the
universe is expanding.
Effect of Doppler shift on spectral lines
The animation below shows how the Doppler Effect can be used to detect
the motion of objects in orbit around one another. This is the
technique used to discover the existence of
exoplanets in orbit around other
stars. Hundreds of exoplanetary systems have been discovered with
this method to date.
Reading for this lecture:
Supplements II and III
Optional reading: Bennett textbook, Ch 5
Reading for next lecture:
Study Guide 11
Bennett textbook: Chapter 7 except pp. 202-212; Chapter 8; "Summary
of Key Concepts" for Chapter 13.
Web Links:
Last modified
December 2020 by rwo
Text copyright © 1978-2020 Robert W. O'Connell. All
rights reserved. Illustrations of blackbody radiation, the 3 types of
spectra, and the solar spectrum copyright © 2000 Harcourt, Inc.
Doppler animation from Dick McCray, University of
Colorado. These notes are intended for the private, noncommercial use
of students enrolled in Astronomy 1210 at the University of Virginia.